Topics and Learning Outcomes
Topics | After reading this Unit and completing the assigned readings in Physical Geology and the associated exercises and questions, you should be able to: |
---|---|
1-1 Introduction |
|
1-2 Minerals |
|
1-3 Igneous rocks |
|
1-4 Volcanism |
|
Reading
Read Chapters 1 to 4 in Physical Geology. You might find it useful to read these chapters section by section as you move through this document. On the other hand, it might be useful to quickly read through each chapter beforehand, and then work through it again as you read through this unit.
1-1 Introduction
Chapter 1 in Physical Geology provides an introduction to the science of geology, which is the systematic study of our Earth. As noted, geology involves a synthesis of knowledge from biology, chemistry, physics, mathematics ,and astronomy, so geologists must have a wide base of understanding. Geologists also must consider an additional factor—the vast amounts of geological time. The significance of this factor cannot be overstated.
As described in Box 1.1 in your textbook, geologists use scientific methods to study the Earth. The key attribute of a scientific enquiry is that it is based on formulating a hypothesis, making predictions that are consistent with this hypothesis, and then gathering data to test these predictions. If the hypothesis and the predictions are well thought out, if the data is carefully collected, and if the results support the predictions, we can say that the hypothesis is reasonable. However, all of these steps together don’t actually prove that a hypothesis is correct, since that would take many similar studies done under different conditions, which all corroborate the initial hypothesis. Two extremely important considerations for doing any kind of enquiry are to avoid jumping to conclusions without carefully assessing the available information, and to remain strongly skeptical about all results—your own and those of others.
Section 1.2 in your textbook includes a list of reasons why it’s important to study and understand the Earth, which are illustrated by the fatal 2005 slope failure in North Vancouver. Another example of the imperative to understand the Earth is related to climate change.
We know the climate is changing because we can see and feel the effects, which temperature records support (Figure 1-1)*. However, to really understand climate change, we need to know how this change compares with natural changes happening now and those that have occurred in the past, and we also need to know about a wide range of both natural and anthropogenic (human caused) factors that can cause the climate to change. Understanding these changes requires careful study of many different types of geological records, plus a strong knowledge of the chemical, physical, biological, and astronomical factors that affect our climate. We’ll look at these factors more closely in Unit 5.
*All figures and tables in these Course Units are labelled with “1-1” style numbers, while those in the textbook use “1.1” style numbers. When referring to figures and tables in the textbook, we’ll always say something like “Figure 2.3 in the textbook.”
Enlarge
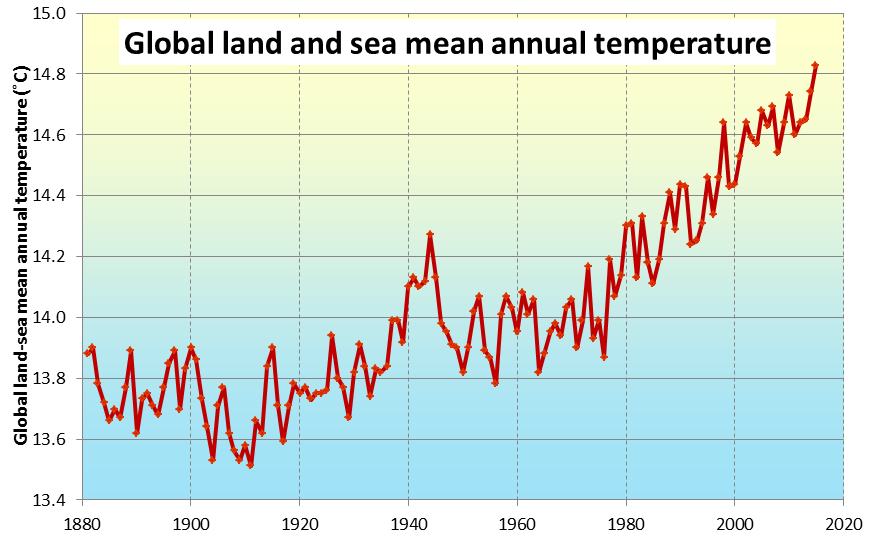
© Steven Earle. Data source: NASA's Goddard Institute for Space Studies (GISS)
Section 1.3 in your textbook includes a very brief discussion of the type of things that geologists do. If you think you might be interested in a career in geology, you’ll probably want to do a lot of reading about other options for geologists and the opportunities for geological work where you live.
Section 1.4 in your textbook is an introduction to the concepts of minerals and rocks. Some of the emphasis is on the difference between minerals and rocks, which is important because we’ll be talking about both at some length during this course. You’ll need to work hard at keeping minerals and rocks separate in your mind, so that when asked, for example, to name a type of rock that might form in a certain setting, you don’t respond with the name of a mineral!
We’ll be looking much more closely at minerals and igneous rocks later in this unit.
Section 1.5 in your textbook includes an introduction to plate tectonics. It’s important to read this part carefully because we’ll be referring to plate tectonics at just about every turn. Understanding the nature of the Earth’s interior is critical to understanding the plate tectonic processes, so look closely at Figure 1.6. We will be studying plate tectonics in much greater detail in Unit 3, but it wouldn’t hurt to have a quick read through Chapter 10 now before going any further.
Physical Geology has numerous built-in exercises to help you engage with concepts, rather than just reading about them. You will be specifically instructed to do some of these exercises within these Course Units, and now is the time to do Exercise 1.2.
For registered students: Exercise 1.1 involves going out and finding a piece of granitic rock. In fact you’re asked to do just that for one of the exercises in Assignment 1, so you should probably go and have a look at the assignment soon*. Some of the exercises require you to answer one or more questions, answers to the assigned exercises can be found at the end of your course textbook.
*Note: If you are a registered student you will need to login to your Learning Management System to access and submit assignments.
Section 1.5 also includes a discussion on geological time. It is critically important for you understand how much time is available, considering the excruciatingly slow pace of many geological processes, because without that understanding, you will have difficulties putting geological events into perspective. When we think of everyday events that are taking a long time to happen, we often say “it’s moving at glacial speed” because it’s well known that glaciers don’t move quickly. It’s true enough that they don’t, but glaciers, which advance at rates of metres to tens of metres per year, are positively speedy compared to most geological processes. Volcanoes, for example, grow at average rates of centimetres per year, less than one-hundredth as fast as glaciers, whereas sediments in lakes or the deep ocean accumulate at tenths of millimetres per year, about one-hundredth as fast as volcanoes grow. Many geological processes are even slower.
Completing Exercises 1.3 and 1.4 will help you with your understanding of geological time, and the notation we use to express past geological times.
Also, work on the questions at the end of the chapter. The answers to these questions are available in Appendix 2 of your textbook. Click on the “Table of Contents” tab at the top-right of any page, and scroll down to the very bottom.
1-2 Minerals
To understand minerals, we need to know something about the bonding between atoms, and to understand that, we need to go back to what an atom looks like, and especially how electrons are arranged around a nucleus. Section 2.1 in your textbook provides a description. If this is review for you, then so much the better. If it isn’t, please read carefully.
OK, now that you’ve done a careful reading, (and without referring back to Table 2.2 in the textbook), consider element number 5: boron (B). How many electrons does it have in the first shell, and how many in the second? How about element number 9: fluorine (F)?
In section 2.2, we look at ionic and covalent bonding. In an ionic bond, electron configuration issues are satisfied by electron transfer. In a covalent bond, electron configuration issues are satisfied by the sharing of electrons.
It’s important to complete Exercise 2.1, and then check your answers on your course textbook.
Section 2.2 also has a discussion on the bonding that occurs within a silica tetrahedron. This bonding is critical because so much of the Earth’s crust and mantle* are made primarily of silicate minerals, all of which include silica tetrahedra bonded in various ways.
The last part of Section 2.2 is a discussion of mineral lattices, which includes a photo of some halite crystals on the left, and broken halite crystals on the right (see Figure 2.8). To refresh your memory, the lattice for halite is shown in Figure 1-2. All of the angles between the atoms are right angles, which helps to explain why halite grows as perfect cubes and also why it breaks along those same planes (known as cleavage planes).
*As illustrated in Figure 1.6, the crust includes only the upper 5 to 50 km of the Earth, whereas the mantle extends from the base of the crust down to the top of the core, which is the greatest volume of the Earth by far.
Enlarge
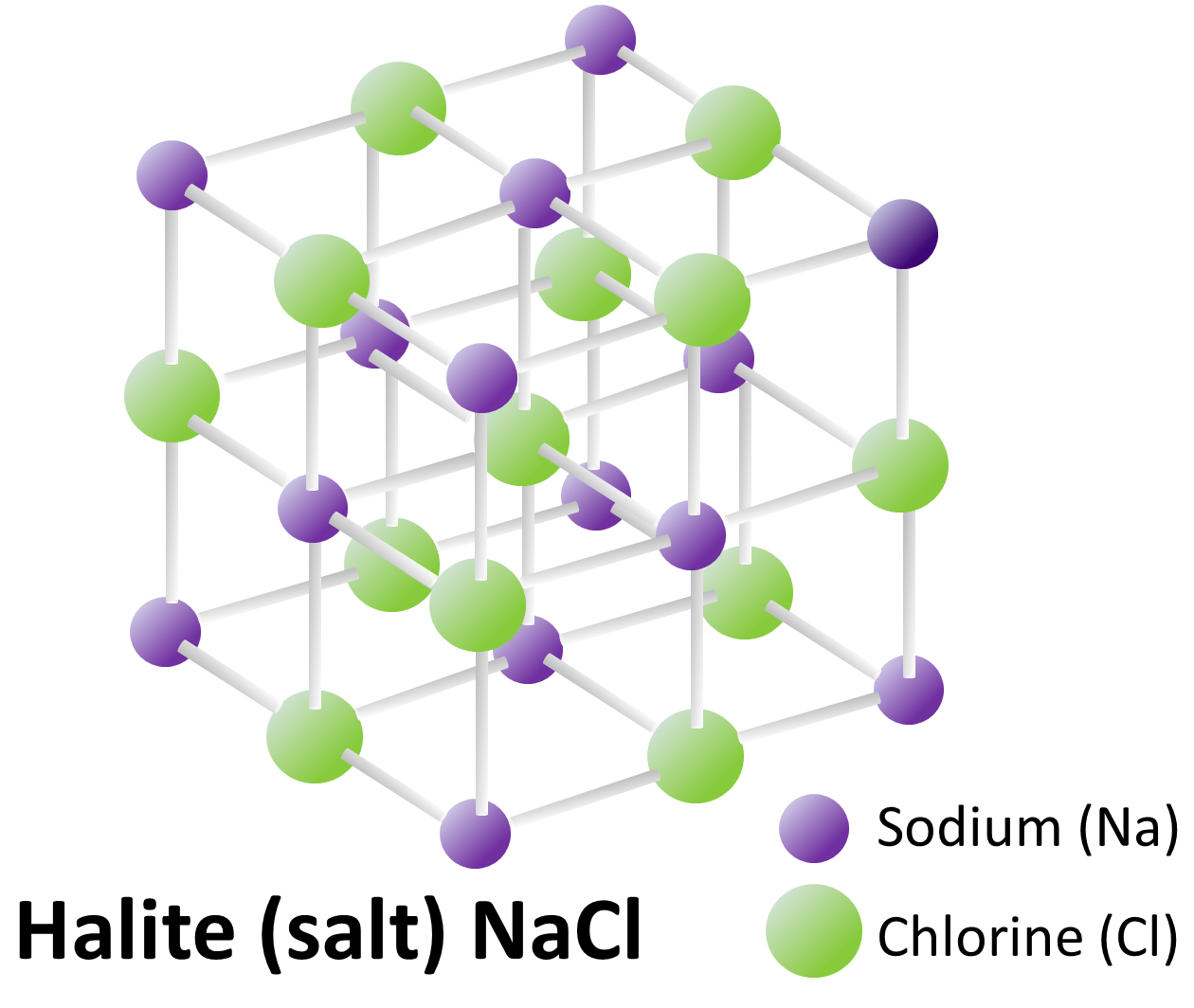
Physical Geology by Steven Earle used under a CC-BY 4.0 international license.
Thousands of different minerals exist, so it’s important to be able to classify them into groups as described in Section 2.3.
To make sure that you understand the rules for classification of minerals into groups, please complete Exercise 2.2.
The important silicate mineral group is described in Section 2.4. As already noted, all silicate minerals include silica tetrahedra arranged in different ways. In some minerals, such as olivine or garnet, the tetrahedra are isolated from each other and bonded to other elements, such as iron or magnesium. In other silicates, the tetrahedra are bonded to each other in a variety of configurations.
Please do Exercise 2.3 to better understand what a tetrahedron looks like. A copy of the figure that you need to cut out is included as Figure 1-3, so just print the page, or click here to access a separate version on its own page.
Enlarge
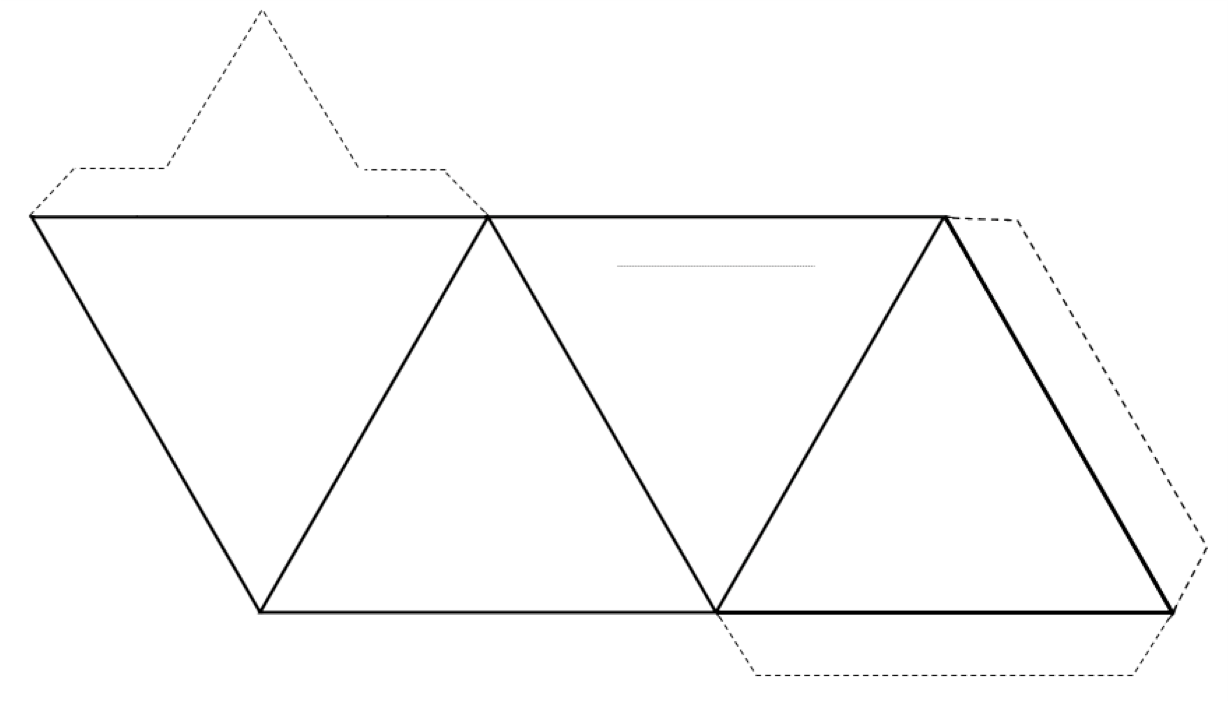
Physical Geology by Steven Earle used under a CC-BY 4.0 international license.
Figure 2.9 in your textbook is a summary of the different arrangements of tetrahedra in silicate minerals, from isolated (e.g., olivine or garnet) to pairs (epidote), rings (tourmaline), single chains (pyroxene), double chains (amphibole), sheets (mica), and 3-dimensional frameworks (feldspar and quartz. Read carefully about these structures, and don’t underestimate the importance of the table of ion sizes (i.e., radii) provided in Figure 2.11. These sizes play important roles in determining which elements will fit into which minerals, and also which elements might substitute for each other in certain elements.Pay special attention to the discussion of feldspars because they are the most common minerals in the Earth’s crust. Feldspars include two main types as illustrated in Figure 2.15. Potassium feldspar includes potassium (K) along with aluminum and silica, whereas the plagioclase feldspars can contain sodium (Na) and calcium (Ca) in varying proportions, along with aluminum and silica. Plagioclase has so many different types because Na and Ca are virtually identical in size (Figure 2.11), and so can easily substitute for each other in the feldspar mineral structure. Potassium, on the other hand, is too large to fit into plagioclase.The nature of silica tetrahedra bonding in silicates not only is important for determining their structure, but also has implications for their chemistry. As described in the textbook, an isolated tetrahedron has 4 oxygens and 1 silicon, and because oxygen ions have a charge of -2 and silicon ions a charge of +4, the tetrahedron has an overall charge of -4 (see Figure 1-4). This strong negative charge has to be balanced by cations such as iron and magnesium, but when tetrahedra are bonded together (in chains or sheets, for example), the oxygens in the corners are shared, which means that some of the oxygens are removed from the structure, and the ratio of silicons to oxygens increases, and fewer cations are needed to create a charge balance.
Completing Exercise 2.4 will help you to understand the concepts of bonding and oxygen sharing in silicate minerals.
Enlarge
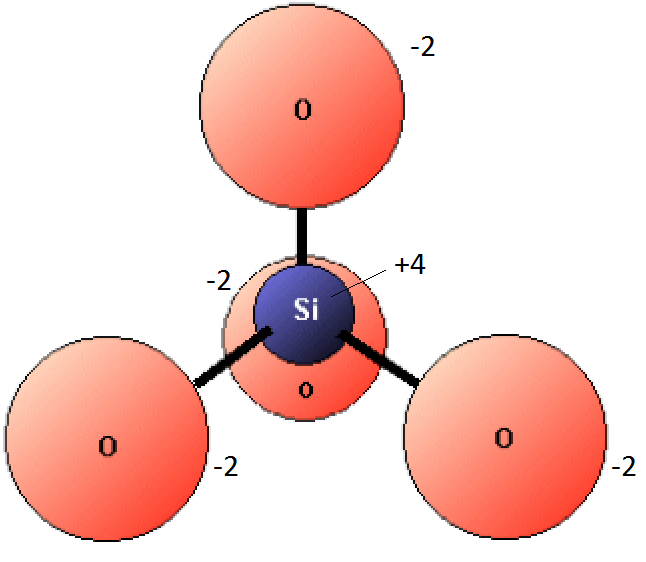
Physical Geology by Steven Earle used under a CC-BY 4.0 international license.
Silicate minerals also are grouped according to whether they contain iron and/or magnesium. Those that do—such as olivine, pyroxene, amphibole, and biotite mica—are called ferromagnesian silicates. They tend to be both darker and heavier than the non-ferromagnesian silicates, which include muscovite mica, the feldspars, and quartz.
Completing Exercise 2.5 will help you to understand the difference between ferromagnesian and non-ferromagnesian silicates. Some of these are tricky, so check your answers on the course textbook.
Section 2.5 provides a summary of the various ways that minerals can form. The vast majority crystallize during the cooling of magma, but some form in other ways.
Note for registered students: In one of the exercises in Assignment 1, you’ll be asked to create some mineral crystals through precipitation from aqueous solution. If you wish, you may get started on that now.
To identify one mineral from another, geologists (and geology students) rely on a series of physical properties that are relatively easy to determine by testing a small sample of a mineral. These properties include colour, streak (the colour of a powder made from the mineral), hardness, habit (the way the mineral grows as crystals), cleavage and fracture (the way the mineral breaks), density, and a few others.
One thing to bear in mind is that these properties apply to minerals, but not to rocks. So if you wanted to test for one of these properties in a rock sample that has visible mineral crystals or grains in it, you have to be careful to actually test the specific mineral of interest.
Please do the questions at the end of Chapter 2 in your textbook. Again, the answers to the questions are available in Appendix 2.
1-3 Intrusive Igneous Rocks
The first part of Chapter 3 in your textbook includes a description of the rock cycle, which is a simple device created to explain the relationships amongst the three main rock types: igneous (rock cooled from magma), sedimentary (rock made up of fragments or dissolved ions derived from existing rock), and metamorphic (rock formed when either sedimentary or metamorphic rocks are heated beyond the stability of their minerals and changed). In the rock cycle diagram (Figure 3.2 in your textbook), two of the important processes are uplift and burial. Uplift is typically achieved by plate tectonic processes, mostly the convergence of plates (moving towards each other) that results in collisions between continents and the formation of mountain ranges. During that process, some rocks get pushed up to form mountain peaks, whereas other rocks get buried deep beneath these mountains. Burial also is achieved in sedimentary environments by the continual process of sedimentation and the very slow build-up of hundreds or thousands of metres of sediments on top of older sediments.
Completing Exercise 3.1 will give you some practice in using the rock cycle, and might also help you to understand the amount of time required for some of these processes to occur.
Section 3.2 describes magma and its formation. Magma is a mixture of elements held at such a high temperature that none of them is able to combine with other elements to form minerals, and so the material is liquid. Temperatures in the order of 800 to 1300˚ C are typical of magmas in the upper part of the crust. Almost all magmas are dominated by the elements shown in Figure 3.6, but the proportions of these can vary quite widely.
All the magma in the Earth’s crust and mantle forms by the process of a partial melting of existing rock as is illustrated in Figure 3.7. As conditions slowly change within the crust or mantle, some of the components of the rock become unstable and start to melt. This partial melting creates a magma that has a different composition than rock—magma always has a higher proportion of silicon (and less iron and magnesium) than the rock that is being melted.
It’s worth repeating here what is stated in the textbook—that contrary to what you might naturally think, a partial melting of the crust and mantle does not normally occur because the rock is heated; instead, partial melting is typically caused by a decrease in pressure (decompression melting) or the addition of a substance (typically water) that has an effect of reducing the melting temperature of the rock (flux melting). These processes are illustrated on Figure 3.8.
Decompression melting is more easily understood than flux melting. Imagine a hot substance that is being kept under pressure. Although the heat makes the atoms move around rapidly to the point where their bonds can break, the pressure contains that effect, keeping them together. When the pressure is reduced, containment is reduced, and some of the substance can melt. Flux melting is based on the principle that a mixture of substances—for example, rock plus water—has a lower melting point than just the rock.
Plate tectonics can account for both of these processes as illustrated in Figure 3.9. Due to the upward movement of mantle material, two processes occur: convection and mantle plumes. As very hot, but still solid rock moves towards surface, the pressure to which it has been subject to decreases immediately, but its temperature decreases very slowly, and the drop in pressure leads to melting. At a subduction zone, oceanic crust is pushed (subducted) down into the mantle. Water from that crust migrates upward into the hot mantle rock, which lowers its melting point and leads to partial melting. This process is shown in a little more detail in Figure 1-5.
Enlarge
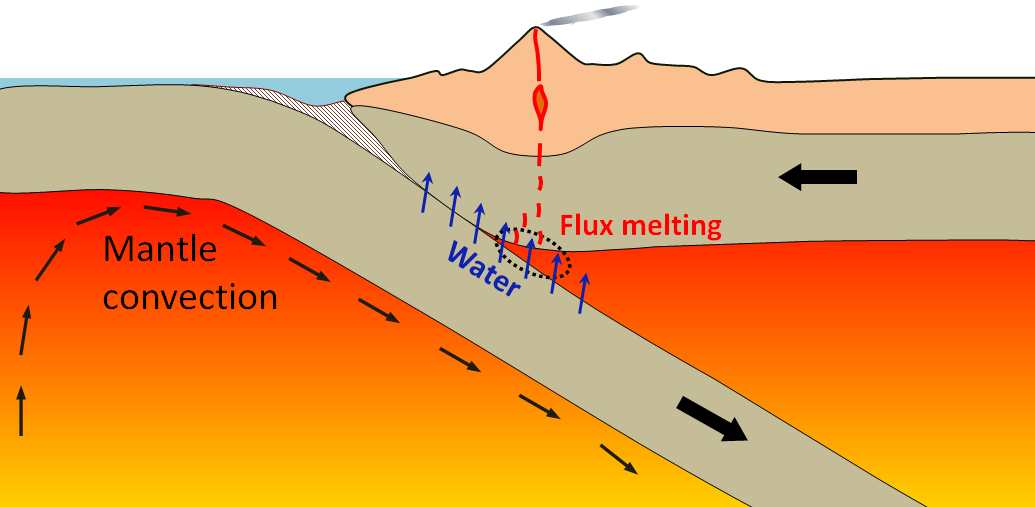
© Steven Earle. Used with permission.
Although we stated earlier that all of the atoms in liquid magma are uncombined, this interpretation is not exactly true. Oxygen and silicon have a very strong affinity for each other, and even while a magma is still very hot, they will start to combine to form silica tetrahedra, and those tetrahedra will start to join together into chains known as polymers. Silica polymers make the magma more viscous (less runny), and the more silica that is in the magma, the more viscous it will be as it starts to cool.
You can experience for yourself how this works by doing Exercise 3.2.
Magma within the upper mantle and crust tends to move slowly towards the surface because it is lighter than the surrounding rock, and eventually this movement reduces its temperature, which leads to crystallization. Like melting, crystallization is a discontinuous and complicated process because although red hot magma may look like a simple liquid—such as liquid candle wax or molten steel—it is very different. As it cools, crystals of different minerals start to form in a generally predictable sequence, which was first described in any detail by Norman Bowen over 100 years ago (see Figure 3.10). The sequence of crystallization depends somewhat on the original composition, but generally it starts with olivine or pyroxene and plagioclase feldspar.
In Section 3.3, carefully read the section on Bowen’s reaction series, since it is a complicated process that has important implications for the textures and mineral compositions of igneous rocks. In the context of this process, make sure you understand the significance of the word reaction.
Magma composition can vary quite widely as illustrated is in Figure 3.12, with the most significant variations being in the proportions of silica, iron, magnesium, and calcium. Magmas (and the rocks that form from them) are classified as felsic if they have more than 65% silica (as SiO2),intermediate if they have between 55 and 65% silica, and mafic if they have between 45 and 55% silica. Mafic magmas have much more iron, magnesium, and calcium—and less sodium and potassium—than felsic magmas.
Completing Exercise 3.3 will help you to understand the differences between mafic, intermediate, and felsic magmas
Figure 3.13 provides a simplified classification of igneous rocks by composition. Using the same three categories—mafic, intermediate and felsic—we can classify coarse-crystalline intrusive igneous rocks as gabbro, diorite and granite respectively, and their fine-crystalline volcanic counterparts as basalt, andesite and rhyolite. By the time you finish this course, you should be very familiar with these six important igneous rock types, and their characteristics.
In many cases, the magma that forms the igneous rocks we see at surface has spent a long time (tens of thousands to millions of years) in a magma chamber within the crust. Some of these processes are illustrated in Figure 3.14. Since temperature increases with depth in the Earth, we can expect that a magma chamber that extends over a few kilometres from bottom to top will be significantly cooler (by as much as 100˚ C) at the top than the bottom. Fractional crystallization is the process during which crystals form in the upper part of the magma chamber and then slowly sink towards the bottom. Since these early-forming crystals, like olivine or pyroxene, tend to be iron- and magnesium-rich, iron and magnesium are removed from the upper part of the chamber and transported to the bottom, where these crystals may re-melt due to the greater heat.
Another process that is not shown in Figure 3.14 is the partial melting of the rock surrounding the magma chamber. Since partial melting favours the melting of silica-rich minerals, such as quartz and feldspar, it typically results in an overall increase in the silica content of the magma.
When a magma cools in two stages—first slow and then much faster—the resulting rock can have a special texture known as porphyritic in which crystals of one or more minerals are much larger than those of the rest of the minerals in the rock (see Figure 1-6 and Figure 3.15 in the textbook).
Complete Exercise 3.4 to gain a better understanding of the process of the formation of porphyritic textures.
Enlarge
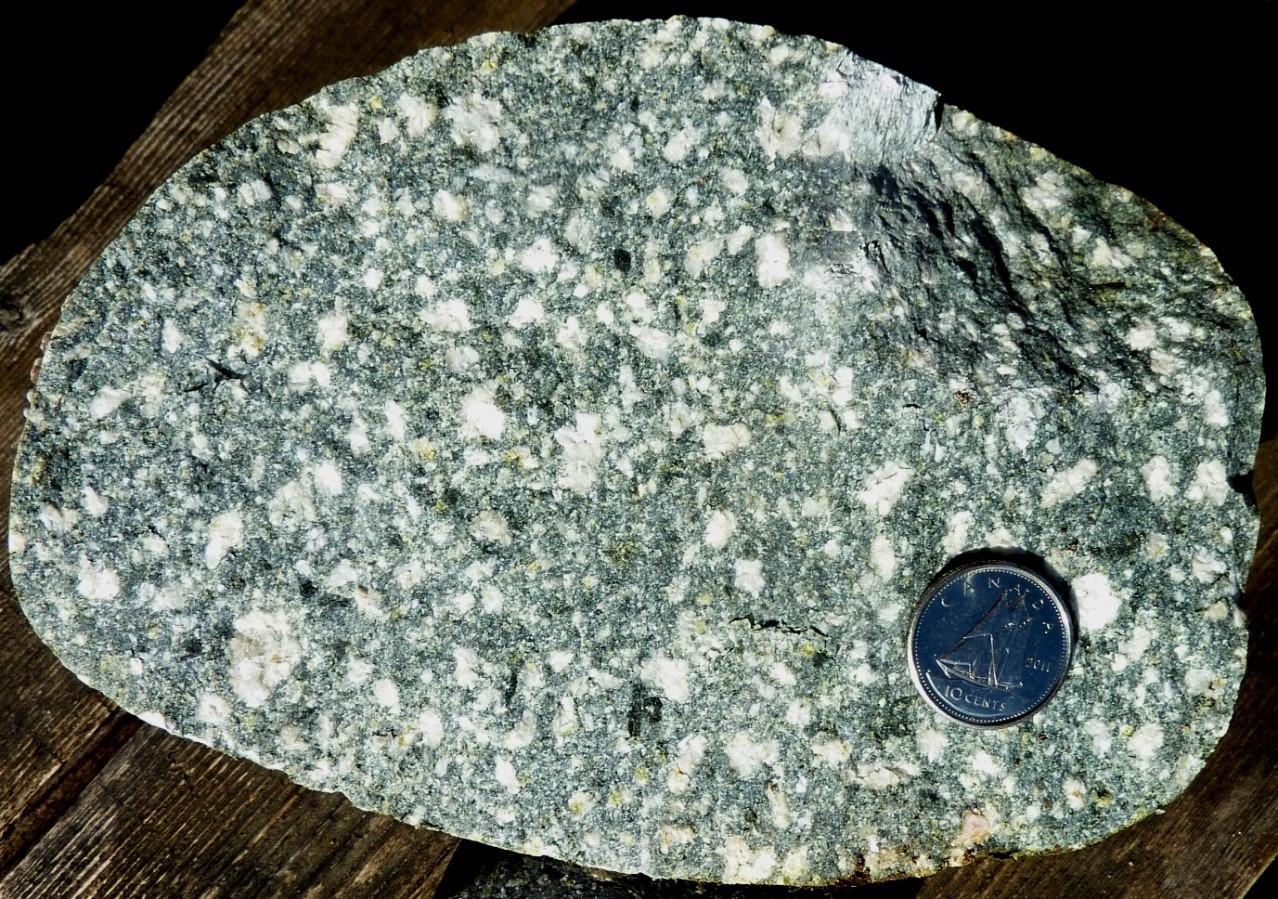
© Steven Earle. Used with permission.
As described in Section 3.4 in your textbook, igneous rocks can be classified according to the proportions of different minerals they contain. In this case, we are talking only about silicate minerals because they make up more than 99% of most igneous rocks. Figure 3.16, one tool for doing these kinds of classifications, shows ferromagnesian silicates in shades of green, and non-ferromagnesian silicates in shades of pink, white, and grey.
Some students have difficulty using this diagram, so it is strongly recommended that you practice with it by completing Exercise 3.5. To help you get started, note that a rock represented by the dotted black line separating “FELSIC” from “INTERMEDIATE” has the following composition: quartz: ~28%, plagioclase feldspar: ~52% (because it extends from 28 to 80%) and biotite/amphibole: ~20% (because it extends from 80 to 100%).
Note for registered students: In the field exercise of Assignment 1, you are asked to find an intrusive igneous rock somewhere in your region, and to describe its texture and estimate the proportions of the different minerals within it. This can be tricky, but you can get some practice with it by doing Exercise 3.6 in which you are asked to estimate only the proportion of the dark (ferromagnesian) minerals, although the same principle can be applied to other minerals.
Igneous rock textures are determined by the rate and conditions of cooling. Rocks that cool slowly have a phaneritic texture with visible crystals (Figure 1-7, left). If the magma is rich in water during crystallization, the crystals can grow very large, which creates a pegmatitic texture as shown in Figure 3.18 in the textbook. When magma cools quickly—typically during a volcanic eruption—the crystals are too small to see (aphanitic texture — Figure 1-7, centre). Some volcanic eruptions are explosive, producing volcanic rock fragments of varying sizes embedded in volcanic ash (pyroclastic texture —Figure 1-7, right).
Enlarge
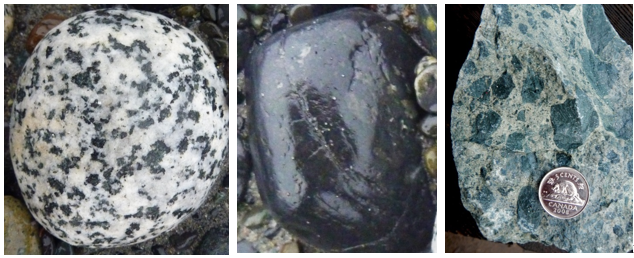
© Steven Earle. Used with permission.
Intrusive igneous rocks are so named because they “intrude” into previously existing rock (known as country rock) and form bodies (known as plutons) with a variety of shapes, sizes, and orientations with respect to the fabric of the country rock.
In Section 3.5, read about dykes, sills, stocks, and batholiths and then do Exercise 3.7 to test your understanding. Some of these identifications are tricky, so check in the course textbook to see if your answers are correct.
Please complete the questions for review at the end of Chapter 3.
1-4 Volcanism
The relationship between volcanism and plate tectonics is described in Section 4.1 in your textbook and illustrated in Figure 4.3. Essentially five different tectonic settings exist in which volcanism can occur, including mantle plumes (also known as hot spots), divergent plate boundaries (also known as oceanic spreading ridges), continental rift zones, oceanic plate-continental plate convergent boundaries, and oceanic plate-oceanic plate convergent boundaries (both also known as subduction zones). In the first three of these settings, the magma originates from the decompression melting of mantle rock. In the subduction zone settings, the magma originates from the flux melting of mantle rock.
As described in the textbook, examples of mantle plume, rifting, and ocean-continent subduction related volcanism are all present in British Columbia. Offshore from Vancouver Island is also an example of volcanism associated with a spreading ridge.
Complete Exercise 4.1 to help you understand how the partial melting of mantle rock leads to the formation of oceanic crust at a spreading ridge.
As explained in the previous chapter in your textbook and also in Section 4.2, when magma is stored in a magma chamber within the crust, a number of processes can lead to changes in its overall composition and also create zonation—more felsic at the top and more mafic at the bottom (see Figure 4.7).
The composition of the magma plays an important role in the style of volcanic eruption that is produced. Although the main factor is magma viscosity, which is controlled by the silica content, the amount of volatiles (gases such as H2O, CO2, SO2, etc.) is also very important, and, as illustrated in Figure 4.8, felsic magmas tend to have more volatiles than mafic magmas.
The pressure exerted by expanding gases as the magma moves towards the surface can make a volcano explode, and you can see how this works by doing Exercise 4.2.
Viscosity is important because while gases can escape (bubble out of) runny non-viscous mafic magmas, they cannot escape easily from viscous intermediate and felsic magmas.
Section 4.3 provides a summary of the main types of volcanoes or volcanic sources. Virtually of the well-known “volcanoes” are either shield or composite volcanoes, and it is important to understand their characteristics and tectonic origins. Cinder cones are small, and it’s not uncommon for them to form on the flanks of larger volcanoes (Figure 1-8).
Enlarge
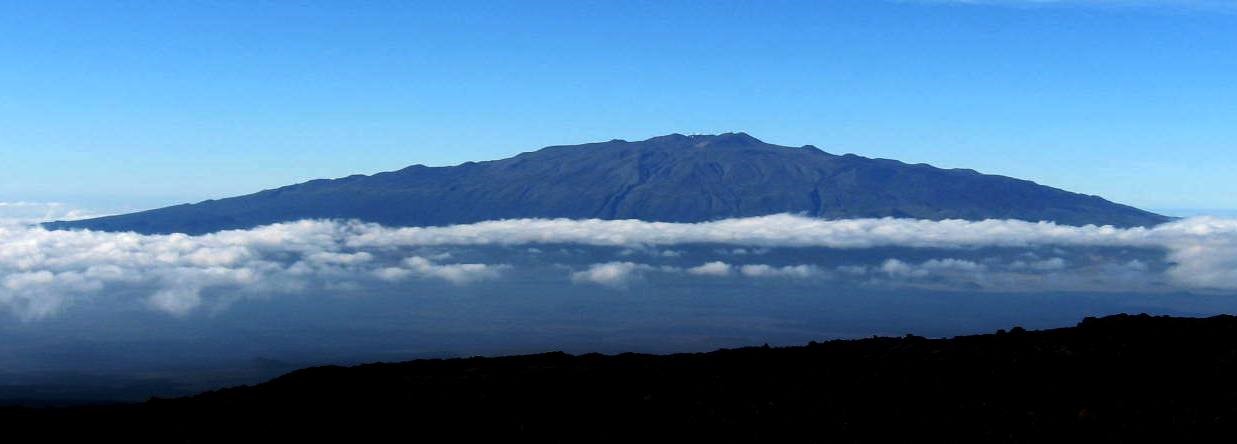
© Steven Earle. Used with permission.
Complete Exercise 4.3, which refers to subduction-related composite volcanoes along the west coast of North America.
Exercise 4.4 is about the ongoing eruption of the Kilauea shield volcano in Hawaii, but if you click on the web link, you’ll see that the “June 27th” (2014) flow didn’t advance much after January 2015. The village of Pahoa is not currently under imminent threat, and the USGS is no longer issuing frequent update maps. The most recent map available (as of May 2016) is included here as Figure 1-9. You can use that to help you answer question 2 in Exercise 4.4.
Enlarge
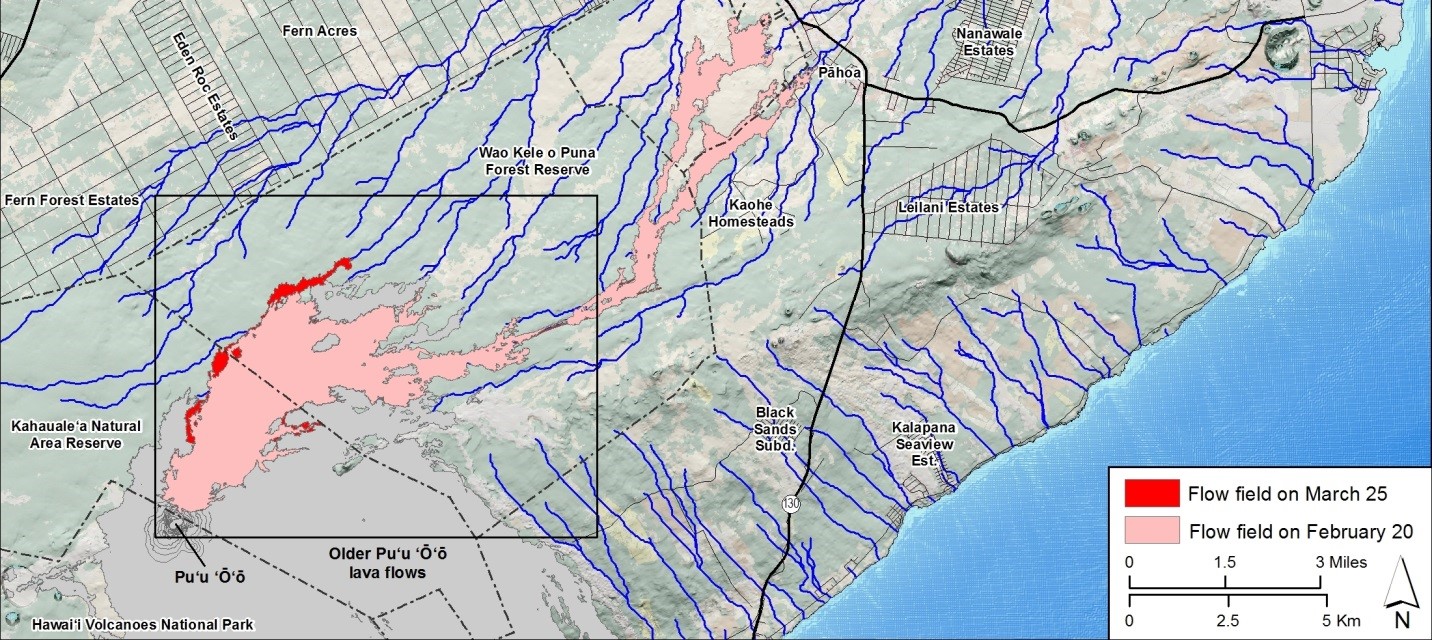
Credit: Department of the Interior/USGS
Basaltic lava flows tend to create two distinctive textures at the surface, which are described by using Hawaiian names. Pahoehoe, or ropy lava, forms when the magma is still very hot and quite runny, and the skin of hardened rock on the surface wrinkles as the flow continues underneath. Aa, or blocky lava, forms when the magma viscosity has increased (because of cooling), but the body is still being forced to move, often because it’s on a steep slope. Both of these textures are illustrated in Figure 4.19. See above to view a short video of a pahoehoe flow at Kilauea in 2007. Please excuse the colourful language!
Large Igneous Provinces are just that—large! The resulting flows cover thousands of square kilometres, and their eruptions are thought to have had major impacts on the Earth’s climate in the past, in some cases leading to major extinctions. As described in the textbook, these large igneous provinces are assumed to derive from the same source as most shield volcanoes—namely mantle plumes.
As summarized in Section 4.4, volcanic eruptions present a variety of hazards, the magnitude of which depends very much on the type and size of the eruption. Some past eruptions have had devastating effects, leading to at least hundreds of thousands of deaths (possibly millions), and they are ranked amongst the deadliest of all natural disasters. The eruptions of the intermediate and felsic magma of composite volcanoes are the most serious direct hazards because they tend to be explosive (due to the high viscosity of the magma), because composite volcanoes are steeper and much less stable than shield volcanoes (so more prone to dramatic failure), and because the eruption cycles are typically centuries apart (allowing entire communities to forget about the hazards and settle on the fertile volcano flanks). On the other hand, some mafic lava flows have had devastating indirect effects. The 1783–1784 eruption at Laki, Iceland cooled the global climate significantly and, according to some estimates, resulted in millions of deaths worldwide, mostly due to famine.
In Section 4.4, read the descriptions of the risks associated with various types of eruptions, and then use Exercise 4.5 to help you understand what you have read.
Although volcanic eruptions have been deadly in historical times, huge advances have been made over the past couple of decades with respect to our ability to monitor volcanoes and predict eruptions, and so thousands of people do not need to be killed by pyroclastic flows, sector collapse, lahars, or ash falls. Modern methods for monitoring volcanoes are described in Section 4.5.
By completing Exercise 4.6, you can apply your knowledge to create a plan for monitoring the Canadian volcano that is most likely to put a large number of people at risk, should it erupt.
As described in Section 4.6, all of Canada’s known volcanoes are in British Columbia and the southernmost part of the Yukon. The most recent eruption was about 250 years ago at the Tseax Cone, north of Terrace, but seismic activity occurred in the area of the Nazko Cone in 2007 that is assumed to have been related to subsurface magma movement, and an eruption occurred at Axial Seamount, off the west coast of Vancouver Island in 2015. Many of the other volcanoes in BC are capable of erupting again in the near future, but you now know how to monitor them if any signs of activity occur.
Please complete the questions at the end of Chapter 4.
This completes the notes for Unit 1.
Note for registered students: If you haven’t already done so, now is the time to start working on Assignment 1 (go to your Learning Managment System). Although you should find most of what you need to complete this assignment within these Unit notes and Chapters 1 to 4 of your textbook, you may also need to refer to other sources of information. If you do use other sources, be sure to document them in your assignment.